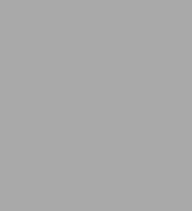
How to Walk on Water and Climb up Walls: Animal Movement and the Robots of the Future
248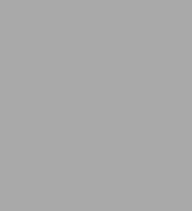
How to Walk on Water and Climb up Walls: Animal Movement and the Robots of the Future
248Paperback
-
PICK UP IN STORECheck Availability at Nearby Stores
Available within 2 business hours
Related collections and offers
Overview
Insects walk on water, snakes slither, and fish swim. Animals move with astounding grace, speed, and versatility: how do they do it, and what can we learn from them? How to Walk on Water and Climb up Walls takes readers on a wondrous journey into the world of animal motion. From basement labs at MIT to the rain forests of Panama, David Hu shows how animals have adapted and evolved to traverse their environments, taking advantage of physical laws with results that are startling and ingenious. In turn, the latest discoveries about animal mechanics are inspiring scientists to invent robots and devices that move with similar elegance and efficiency. Integrating biology, engineering, physics, and robotics, How to Walk on Water and Climb up Walls demystifies the remarkable secrets behind animal locomotion.
Product Details
ISBN-13: | 9780691204161 |
---|---|
Publisher: | Princeton University Press |
Publication date: | 03/03/2020 |
Pages: | 248 |
Sales rank: | 118,683 |
Product dimensions: | 5.20(w) x 7.90(h) x 0.80(d) |
About the Author
Read an Excerpt
CHAPTER 1
Walking on Water
On calm ponds and rivers, long-legged insects stand on the water surface as if it is land (Plate 2). They are the water striders, and in this chapter, we'll learn how their motion has inspired a water-walking robot. From above, the bodies of water striders are dark brown and kayak-shaped, streamlined for speed. Although they stand quite still most of the time, at the first sign of an intruder, they seem to just disappear, generating the equivalent of a sonic boom on the water surface, an explosion of small water waves radiating outward behind them. How can they stand on water when we cannot?
Water striders can stand on water because their small size allows them to take advantage of surface tension forces. Conversely, at your body size, the surface of water appears quite weak. You can step into a bathtub or stick straws into a drink without a second thought. However, water striders are small and light enough that they are sensitive to forces that you would find negligible. Specifically, they exploit surface tension forces, which arise due to the attraction of water molecules to each other. It takes energy to push these molecules apart, or to increase the water's surface area. Because of surface tension, bubbles are round to minimize their surface area. At the same time, a water strider's low weight of only 10 milligrams, or just three sesame seeds, is enough to bend but not break water surfaces. Surface tension supports the water strider's weight just like a trampoline supports yours (Fig. 1.1). That water striders experiences a different world than you do because of the physical consequences of scaling. Simply put, the forces acting on an animal depend upon its body size. We'll see this principle at play throughout this book, but it especially makes a difference for small animals.
If you were to try walk on water like a water strider, your body weight, about 10,00,000 times that of a water strider, would require feet nearly 10 kilometers across to be supported by surface tension. No one has ever built a foot that big, but there have been attempts to make shoes the size of kayaks, which are supported by the same buoyant force that prevents boats from sinking. When such buoyant forces act on feet, the result is often ungainly. In annual competitions where students wear boats on their feet and attempt to walk on water, forward motion is at best two steps forward, one step back.
I was first introduced to water striders by John Bush, a mathematician who at the time was one of the world's experts in fluid flows driven by surface tension. In the fall of 2001, I enrolled in his introductory course in fluid mechanics, which culminated in a final course project of the student's own design. When I came to his office one day, he took a book off his shelf and pointed to a page that stated that infant water striders shouldn't be able to move. He was confident that it was a fallacy, and he suggested that for my course project, I try to unravel how infant water striders could propel themselves. Hunting down water striders to understand their motion didn't sound like research. It sounded like fun.
Seeing them in person was quite different from reading about them in a book. I tagged along with a group from my dormitory that was traveling to Walden Pond for a picnic. I used nets to hunt and catch the water striders. I kept them on top of damp paper towels, in the sandwich boxes that I had brought my lunch in. When I returned to John's lab, I dropped the striders into an aquarium full of water. They fell like feathers onto the water surface. As they stood on the water surface they used their long legs to groom themselves, rubbing as if playing the violin.
In the glass aquarium, I could see their legs from below (Plate 3). They looked like rowboats with six oars on the water. When the oars rested on the water surface, they were coated in a silvery layer of air, reflecting light like a diamond. If you were to submerge your own hand underwater, such a sparkling air layer would be absent. The water strider traps such a layer because it has the hairiest legs in the world, legs with 10,000 hairs per square millimeter, a million times denser than the hairs on a human head. Moreover, each of these hairs is covered in an array of grooves that make it even more water-repellent. The water strider's hairs, or trichia, were first pointed out by French and Danish naturalists in the 1950s. In 2004, Chinese chemists built a synthetic water strider leg by depositing fatty chemicals onto a smooth quartz fiber. Their synthetic leg could support the force of a water strider standing, but not the larger forces of gliding or jumping. The hairs on the water strider's legs thus have an important function in augmenting its water-repellency.
Although the functioning of the ornate details of the water strider leg remain under contention, one thing is clear: these hairs keep the strider dry by increasing the surface area of the leg. This is an interesting feature of surfaces. Using fractals or other types of patterns, one can increase the surface area of an object indefinitely. When such a large surface area is coated with a waxy water-repellent coating, water applied to the leg is kept at bay. Looking closely at the leg when it stands on the water surface, the water holds station at the tips of the hairs. It cannot penetrate the small grooves between the hairs. The result is that the water strider stands on water by standing on air.
The air layer on their legs gives water striders a remarkable ability, to glide on water for long distances, as if they are skating on ice. This ability has given the striders another name, pond skaters. During a rowing stroke, adult water striders clock up to 50 body lengths per second, the equivalent of a human running a 100meter dash in a single second. After this high-speed stroke, they glide effortlessly for over ten body lengths. This gliding can be easily triggered: simply blow on a water strider. Like a puck on an air hockey table, the strider seems to just glide and glide. But if it glides so well, how does a water strider start moving in the first place? Where does the initial traction come from? The first person to recognize this problem was Stanford biologist Mark Denny, who wrote about it in his book on biomechanics in 1993. He came to the startling conclusion that he could not explain how water striders propelled themselves.
Mark proposed that the water surface changes state depending on how it is pushed. When the water strider glides, the surface is smooth like a linoleum floor. But when it rows backward, the water surface ruffles up like a trampoline, resisting the leg's motion. This resistance, the generation of a wave behind the strider, is enough to push the strider forward, so Mark thought.
Mark's idea of wave-based propulsion may work for the larger water-walkers, but for infant water striders it was problematic. Hydrodynamic theory states that surface waves can only be generated if an animal can move its legs fast enough. For straight-line motion at constant speed, that minimum speed is 23 centimeters per second, a low enough speed that it can be tested in the bathtub or swimming pool. If you dip a finger into the water and push it like a small boat, you do not create waves unless you travel faster than this minimum speed. While adult water striders have legs a centimeter long, infant water striders are ten times smaller, and their legs are barely one millimeter long. For infant water striders to achieve the required speed, they have to rotate their legs at rates higher than 1000 cycles per second, or 500 times faster than we can pedal on a bike. An infant strider trying to achieve these speeds would likely injure itself. The question of how infant water striders could move became known as Denny's Paradox, named after Mark Denny.
Denny's Paradox, and the idea that waves were necessary for water striders to move, spread across scientific zip codes. In Poughkeepsie, New York, Vassar biologist Robert Suter studied water spiders, thick-legged cousins of the striders. Suter observed that spiders also generated waves as they moved on the water surface. Even an isolated leg of a water spider, suspended in a flowing water tunnel, generated waves in its wake. Mathematicians from Stanford University calculated the wave field generated by a water strider's legs. The idea that waves were necessary for motion on the water surface became increasingly accepted by both mathematicians and biologists. But the more scientists accepted Denny's wave theory, the more puzzling Denny's Paradox became.
I had taken some photos of water striders for my project in John Bush's class, but I still didn't have an answer to Denny's Paradox. Resolving the paradox would require teamwork and some high-tech equipment so we could see more clearly what the leg was doing. I teamed up with my classmate Brian Chan, a mechanical engineer who was eager to build a robotic water strider. Satisfied with my performance in his course, John gave me the combination to the door to his basement lab, which was called the MIT Applied Mathematics Lab, a place where mathematicians did experiments with different kinds of liquids. It was filled with cameras and shelves of strange-shaped transparent vessels through which liquids could flow. At once, I had a PhD advisor and my own place to do research. My education in fluid mechanics had begun.
With the water striders now in the lab, we borrowed a high-speed camera from the Edgerton Center across campus. Our first high-speed videos of water striders showed quite a different world than what we could see with our eyes. The entire rowing stroke took a hundredth of a second. You could fit 30 rowing strokes into a single eye blink. With the camera, we saw that water striders rowed their middle legs with such force that their body was rocketed forward and upward into the air. Consistent with Mark Denny's predictions, the water surface behind ruffled up like a trampoline.
Denny's Paradox told us that the ruffled trampoline we saw could not be the only force driving water striders forward. The water striders were clearly getting thrust somewhere else, but where? We turned to flow visualization, the use of particles or dyes to pinpoint the motion of fluid. It's a technique that dates back hundreds of years, when visualizations of flying pigeons were made by throwing sawdust up into the air. As pigeons flew through, the falling sawdust spun into vortices. The strategy is to keep most of the fluid transparent, and place tracer particles in the region of interest. For the water striders, we needed a technique that stayed close to the action, the water surface. John knew of a technique used to visualize the impact of vortices using the chemical thymol blue. Thymol blue is a pH-activated dye, originally intended for chemistry experiments to show when a reaction was complete. We had not heard of it being used with live animals before, but why not?
In the lab, we began using the thymol blue to visualize the flows generated by the water strider's leg stroke. We first made the water alkaline by dissolving sodium hydroxide pellets, which look like rock sugar. We placed water striders on the water surface, and lit them from below using the kind of light box children use with tracing paper. Then we sprinkled in the dye with our fingers, like dried oregano. Sometimes a few flakes fell on the striders and they brushed themselves off, as if they were flicking off falling snowflakes. When the flakes landed on the water surface, the water blossomed into a deep blue color with small hints of yellow. When the striders were blown gently, they rowed, and the dye began to trace out their wake (Fig. 1.2, Plate 4).
With the aid of the thymol blue, we saw that the waves generated were only a brief manifestation of the rowing stroke. The waves came and were gone. Instead, what attracted our eyes was a butterfly-shaped dipolar vortex behind each leg. In general, a vortex is a spinning region of fluid, similar to the one generated in a cup of coffee when you stir it with a spoon. A dipolar vortex is generated from the rowing motion of an oar and has the shape of a palmier cookie. Even infant water striders were capable of generating vortices. The presence of such vortices provided a resolution to Denny's Paradox. By measuring their size and speed, we found they contained as much momentum as the water strider. The forward movement of the strider, like that of a fish, relies on pushing a packet of fluid backward.
Animals that propel themselves by pushing fluid backward must satisfy conservation of momentum. Consider a hummingbird that flaps its wings so that it hovers in midair. To hold station, it must constantly throw air downward. If the bird stops doing so, it sinks. Since air is so light, weighing 1000 times less than water, the bird must throw air at quite a high rate. The air that is thrown down has a certain momentum, the product of its mass and speed. The rate that momentum is thrown downward must be equal to the bird's weight for it to hover. A helicopter works the same way: its rotors push on air, speeding it up, increasing its momentum, and then throw the air downward as frequently as they can.
For a fish to swim forward, it must also satisfy conservation of momentum. As the fish moves forward, it flicks its fins and tail to generate an approximately fish-sized wake traveling in the opposite direction. Conservation of momentum dictates that this wake travels in the opposite direction of the fish. The form of the wake often depends on the mode of locomotion used. A tail fin generates a series of linked vortices like links of a chain. Birds flying at low speeds create a vortex with each flap, generating a series of vortex rings, like smoke rings. The wake of a water-running basilisk lizard consists of vortices that travel both downward to support its weight, and backward to enable it to generate thrust. The generation of vortices is a hallmark of the motion of many animals in water. Water striders live atop water, not in it, and it is likely for that reason that no one until John Bush had suspected they could generate vortices. Despite their sitting at the surface of water, water striders join the ranks of animals like birds, fish, and lizards that all create vortices to propel themselves.
If you have seen a water strider, you might be surprised that it can create vortices as large as watermelon seeds. A water strider is like a rower who has chopped off the blades of its oars and simply uses two thin sticks to row. In this case, its legs are fifty times thinner in diameter than the width of the vortex. How can such legs move the fluid? The answer is surface tension, but it arises in a subtle way. As a water strider sits on the water surface, it creates dimples, deformations of the water surface. These dimples look like small magnifying lenses centered on the strider's legs tips. The dimples are retained as the strider rows its legs. Filled with air but held together by surface tension, the dimples are like paddles that can be used to catch and push on more water than the insect could with just its spindly legs alone. Thus, water striders use their legs as shafts, their dimples as blades. The explanation was so elegant that we could hardly believe it. We had to try it out ourselves.
As autumn stretched into winter, water striders could no longer be found outside, but my classmate Brian had become a bit obsessed with them. One day, as we stopped to chat in the hallway, he pulled out the beaten notebook he carried around in his jacket pocket and showed me his drawings. They were a mix of mathematical equations and sketches of water striders of all shapes and sizes on the water surface.
The hardest part about building a robotic water strider is making it lightweight enough to balance on water. It's a tough constraint far beyond the capability of most robots. One of the most recognizable humanoid robots is Honda's walking robot called Asimo. It is four feet tall and weighs 120 pounds. Steel is heavy, and batteries, composed of metal, lead, and liquid chemicals, even heavier. In contrast, a typical water strider weighs a hundredth of a paperclip.
(Continues…)
Excerpted from "How to Walk on Water and Climb up Walls"
by .
Copyright © 2018 Princeton University Press.
Excerpted by permission of PRINCETON UNIVERSITY PRESS.
All rights reserved. No part of this excerpt may be reproduced or reprinted without permission in writing from the publisher.
Excerpts are provided by Dial-A-Book Inc. solely for the personal use of visitors to this web site.
Table of Contents
Acknowledgments ix
Introduction The World of Animal Motion 1
Chapter 1 Walking on Water 16
Chapter 2 Swimming under Sand 32
Chapter 3 The Shape of a Flying Snake 60
Chapter 4 Of Eyelashes and Sharkskin 88
Chapter 5 Dead Fish Swimming 114
Chapter 6 Flying in the Rain 139
Chapter 7 The Brain behind the Brawn 155
Chapter 8 Are Ants a Fluid or a Solid? 179
Conclusion The Future 203
Bibliography 215
Index 221
Plates follow page 138
What People are Saying About This
"Answers questions you probably won’t realize you even had. . . . Hu demonstrates the extraordinary value day-to-day curiosity brings to science."—Christopher Intagliata, Science Friday"[Hu] describes both the silliness and profundity of his brand of research. . . . Middle school science teachers and nerds everywhere will thank him."—James Gorman, New York Times"[An] engrossing tour of faunal motion."—Barbara Kiser, Nature"Hu distills the complex science that demystifies how flying snakes glide and sharks make for remarkably efficient swimmers. Breaking down these concepts is not easy and Hu is up to the task."—Ronak Gupta, The Wire"This highly accessible and exciting book is a quick, enjoyable adventure."—Grrl Scientist, Forbes